What makes up the grid?
- Power plants
- High and low-voltage transmission lines, which transport power over long distances Substations for routing power on the transmission grid
- Transformers that change voltages on the distribution grid / Distribution lines, which bring power directly to buildings or other end points
- Substations on the distribution grid / Transformers that change voltages on the distribution grid
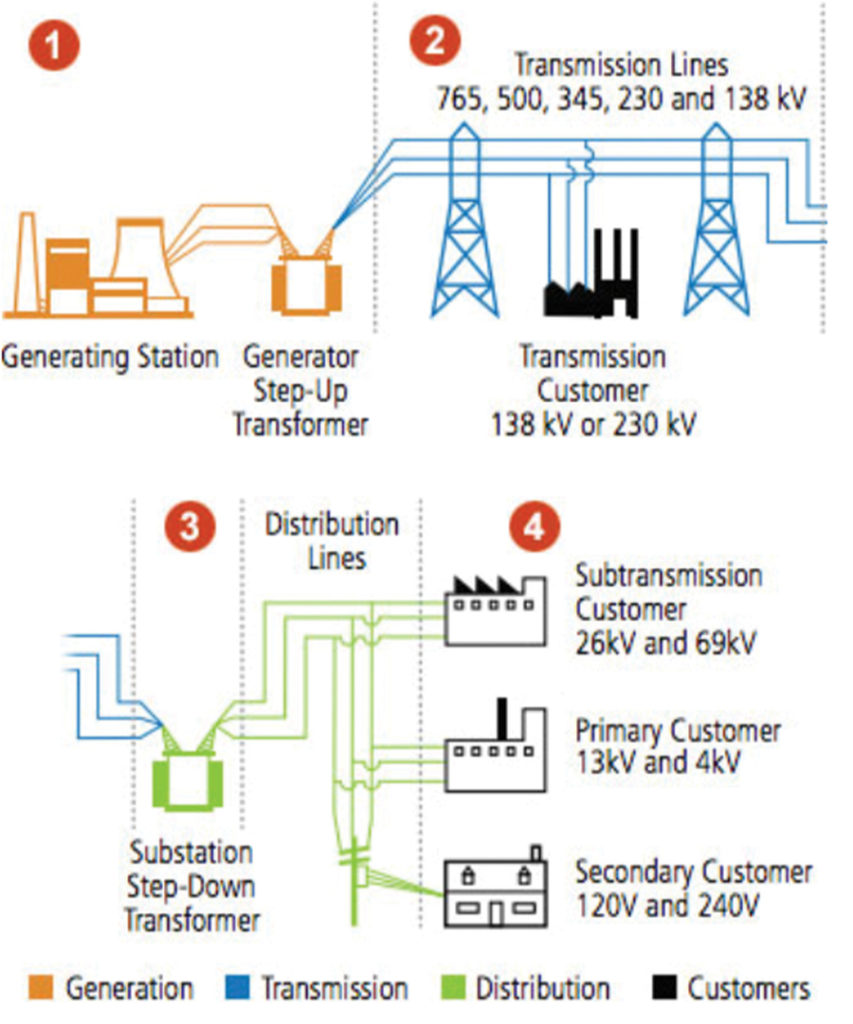
What Our Energy Grid looks like right now:
- Solar – 27.2 gigawatts of solar power capacity [enough power to supply about 6 million homes]
- Coal – 285 gigawatts of coal capacity (one plus is that it is the cheapest, only $25 per megawatt hour]
- Natural gas – 440 gigawatts [see a capacity of 440 gigawatts]
- Nuclear capacity – 98 gigawatts
- Hydroelectric capacity – 80 gigawatts
- Wind power – 74 gigawatts [see 74 gigawatts of installed capacity]
TOTAL = 1004.2 Gigawatts (current estimate is 1,100 GW)
Our Goal:
- Start building solar industrial plants at 50 Giga watts per year – 400 Gw in an “8 year plan” [typical plants are 600 MW, would need 80 new plants]
- Hydro potential probably is maxed out at 140 gigawatts (currently 80 Gw), so 64 Gw potential or 8 Gw per year in our “8 year plan”
- Nuclear sits at 98 Gw and will have 8 years to get its shit together and help us out
- We will try to get Wind Power from 74 to 200 Gigawatts, or an increase of 16 Gw capacity per year for 8 years
After 8 years, we would have:
- 400 Gw solar
- 200 Gw wind
- 140 Gw hydro
TOTAL = 740 Gw, meaning we need nuclear to increase to as much as 300 Gw, or combine hydrogen with it – either way, We need perhaps 300 Gw from some other source (Smart Roads / Methane to Energy Conversion / ocean waves / tides / thermal / hydro power from new large water pipes can help, too).
THE BASIC PLAN:
- Build solar (50 Gw / yr), wind (16 Gw / yr) and hydro (8 – 10 Gw / yr)
- Put $$$ into nuclear (better hurry up Nuclear!) if they don’t get it together in 8 years, We will stop funding them and continue with other viable sources.
- We need a storage battery business – to store solar and wind for the grid, and for our cars, to make them cheaper.
- Build Battery Charging Stations for our Electric Vehicles [that We might also need to manufacture].
- Go into the solar powered hydrogen producing fuel station business.
- Go into both Hydrogen fuel cell vehicles, and the gas-powered to Hydrogen fuel cell powered car conversion business.
- We will attempt to change our lifestyle in order to use less electricity (this remains to be seen, but we must always attack a problem from every angle).
- And of course we need a massive new Power Grid.
SOLAR POWER
Highest statewide average solar voltaic capacity factors are in Arizona, New Mexico, and Nevada (each 26.3 percent); the lowest is Alaska (10.5 %). The lowest statewide average capacity factor in the contiguous 48 states is in West Virginia (17.2 %).
This intimates that we need to install 4 Gw capacity in the sunny states to get at least 1 Gw of actual electricity, in Alaska we need to install a 10 Gw capacity installation. We will need 100 sq ft for every 1kW of solar panels. Extrapolating this, a 1 MW solar PV power plant should require 100,000 sq ft (about 2.5 acres, or 1 hectare) [this is modeling for about 5 hours of sunlight per day – to generate 1 MW for 24 hours would take 4x the amount of space (and panels)].
Thus: The equivalent of a 1 MW power plant would require 2-2.5 acres of land, nearly 14,000 solar panels and a ton of batteries. We are told to “Assume that a 1 MW solar farm would cost roughly $1 million to install”.
MATH:
125,000 acres, $50 Billion, 700 million panels per year
- federal land
- panel making industry
- Our business needs to make everything – mono crystalline solar cells, etc – we cannot pollute while doing it, so R & D may be needed
- panel installing industry
- the $50 billion Bank Loan to pay for it
Trina Solar is a good model – it makes everything in-house – 5 Gw production capacity is now the norm.
SunPower produces the highest efficiency monocrystalline solar panels available. “Our X22 has a record-breaking efficiency of up to 22.8 percent, making it the best performing panel on the market today. “[Polycrystalline panel efficiency typically ranges from 15 to 17 percent.]
Hanwha Q Cells is China’s biggest solar cell manufacturer, and it really makes them cheap – $2 billion a year business – The company develops and produces crystalline silicon photovoltaic cells, panels, and silicon ingots and wafers. It has 1,350 employees, and 5.5 Gw capacity in solar panel production.
Currently in US – 4.4 GW of crystalline silicon module capacity at the end of 2019, if all factories are completed on schedule.
TAKEAWAY:
We are way short of where we need to be, so guess what, we’re going into the solar cell business.
SunPower uses Maxeon solar cells – Maxeon® solar cells, delivering up to 22.7% efficiency and up to 370-watt residential panels. Perfect for space constrained projects. Larger panels for commercial use range up to 470 watts per panel. Our business needs to make everything – mono crystalline solar cells, panels, etc.
WIND POWER
Wind Turbines – Over the course of a year, turbines will typically generate about 24% of the theoretical maximum output (41% offshore). This is known as its capacity factor. An average onshore wind turbine with a capacity of 2.5–3 MW can produce more than 6 million kWh in a year. In the United States, the direct land use for wind turbines comes in at three-quarters of an acre per megawatt of rated capacity. That is, a 2-megawatt wind turbine would require 1.5 acres of land.
MATH:
- 2 MW turbines takes 1.5 acres. We need 16 Gw, which is 8000 new turbines a year at $3 million per turbine. This translates into 12,000 acres, $24 Billion Bank Loan
NOTE: Our Affordable Housing needs to pitch in on the Energy Production, too
- Wind Trees – Installed power – 5.4 kW, 2400kwh a year. But probably not Solar Panels. *
* If you compare a large industrial installation made up of solar panels on racks that are organized to point to the sun accurately and track the sun, the cost is 2 to 3 times lower than those same panels installed on a typical rooftop. Sharing is better – in economics, we’re much better off if we share resources than if each person has all the resources themselves.
BOTTOM LINE: When you put solar panels on your rooftop, you feel autonomous, which feels good, like you are doing something, but in reality, your neighbor’s taxes are paying for you to be autonomous – once again we are duped into thinking we are paying the actual cost of things, but it isn’t true.
HYDRO-ELECTRIC POWER
“U.S. Department of Energy and the Oak Ridge National Laboratory released a hydropower mapping tool in 2014 that estimates more than 65 GW of potential new hydropower development across more than three million U.S. rivers and streams that currently do not have hydropower development [13]. The study identified western states, including Alaska, California, Colorado, Idaho, Montana, Oregon, and Washington as having the greatest new hydropower development potential.
MATH:
Hydro comes in many types and sizes, but basic construction cost is $580 per kilowatt of installed capacity. We need 8 Gigawatts a year, which would cost us a $4.64 Billion Bank Loan.
NOTE:
Garney Construction is in charge 142 miles of pipeline in San Antonio. They are working on an idea to put hydropower turbines in the very largest water pipes to create power while water is being delivered. Clever. Don’t we need some new Water Infrastructure?
NUCLEAR POWER
If it happens, we would need $5,339/Kw to build. Current Plants range from 582 Mw to 3,937 Mw electricity generating capacity. We might eventually need up to 300 Gw, so would be best to shoot for building 50 Gw per year.
MATH:
$5,339 (per Kw) X 50 million (Kw’s) = $267 Billion Bank Loan
WHERE WE ARE RIGHT NOW
As of today, there are more than 700,000 MT of depleted uranium in the United States [10]. This is a huge reserve that, with B&B reactor technology, can be directly used for energy production.
3 Designs of Breed and Burn reactors: Tokyo Institute of Technology developed several conceptual designs of the B&B reactors, called CANDLE. The CANDLE reactor uses an axial fuel reload scheme and needs a high requirement of fuel burn-up (∼ 40%). In 2008, a private company, TerraPower LLC was founded with the goal of commercializing B&B technology and building a prototype by 2020. Later, Greenspan et al. at University of California, Berkeley, developed several designs of the Breed and Burn reactors where the fuel is shuffled radially with a cylindrical arrangement of hexagonal fuel assemblies.
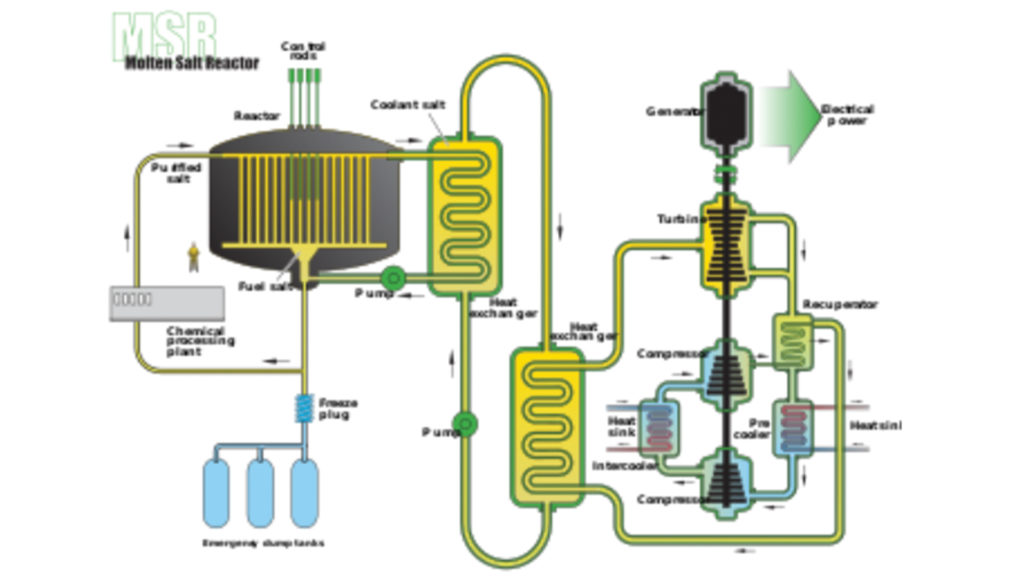
Small modular reactors [SMRs] NuScale (which has already submitted its design to the feds), are trying to develop light-water SMRs. Their designs use the light water approach to cooling and power generation that is common in conventional nuclear plants. Water is heated by the nuclear core at the base of the reactor vessel, which flows upwards inside the riser, then down over steam generators. As heat is transferred to steam generators, the water becomes cooler and denser, sinking back to the bottom of the device, where the cycle is repeated. Steam generators turn a turbine, which drives an electrical generator. Alternative cooling systems using molten metals are expected to operate at even higher, more efficient temperatures once approved.
ISSUE: require refueling with standard 4.95 percent enriched uranium-235 fuel every two years.
Molten Salt Fast Reactors (MSFRs) exhibit large negative temperature and void reactivity effects. These large negative effects lead to a favorable, much more stable operational behavior than in classical fast reactors with solid fuel. The strong negative feedback effects are a unique safety characteristic which is not found in any kind of solid-fueled fast reactors [8] and lead consequently to superior inherent safety characteristics. MSFR systems have been recognized as a long-term alternative to solid-fueled fast-neutron systems due to several additional unique favorable features (smaller fissile inventory, easy in- service inspection, simplified fuel cycle, etc.)
Liquid fluoride thorium reactors are designed to be meltdown proof. A plug at the bottom of the reactor melts in the event of a power failure or if temperatures exceed a set limit, draining the fuel into an underground tank for safe storage. Mining thorium is safer and more efficient than mining uranium. Why
We like Molten Salt Reactors:
- MSRs are walk-away safe. Even a human engineered breach (such as a terrorist attack) of an MSR cannot cause any significant release of radioactivity.
- Radioactive byproducts of fission like iodine-131, cesium-134 and cesium-137 (such as those released into the atmosphere and ocean by the Fukushima meltdown) are physically bound to the hardened coolant and do not leave the reactor site.
- Not only do MSRs not have a long-term waste issue, they can be used to dispose of current stockpiles of nuclear waste by using those stockpiles as fuel. Even stockpiles of plutonium can be disposed of this way.
- Conventional reactors typically use only 3-to-5% of the available energy in their fuel rods before the fuel rods must be replaced because of cracking. MSRs can use up most of the rest of the available fuel in these rods to make electricity.
- Although it is sometimes claimed that nuclear power is not sustainable, the truth is that there is enough nuclear fuel on earth to provide humanity with abundant energy for millions of years.
- A variant of an MSR, a liquid fluoride thorium reactor (LFTR), will be able to use abundant thorium as a fuel.
- In addition, breeder reactors (which include some types of MSRs) make it possible to use uranium-238 as fuel, which makes up 93.3% of all natural uranium. Conventional reactors use only uranium-235, which makes up a mere 0.7% of natural uranium.
Battery Storage
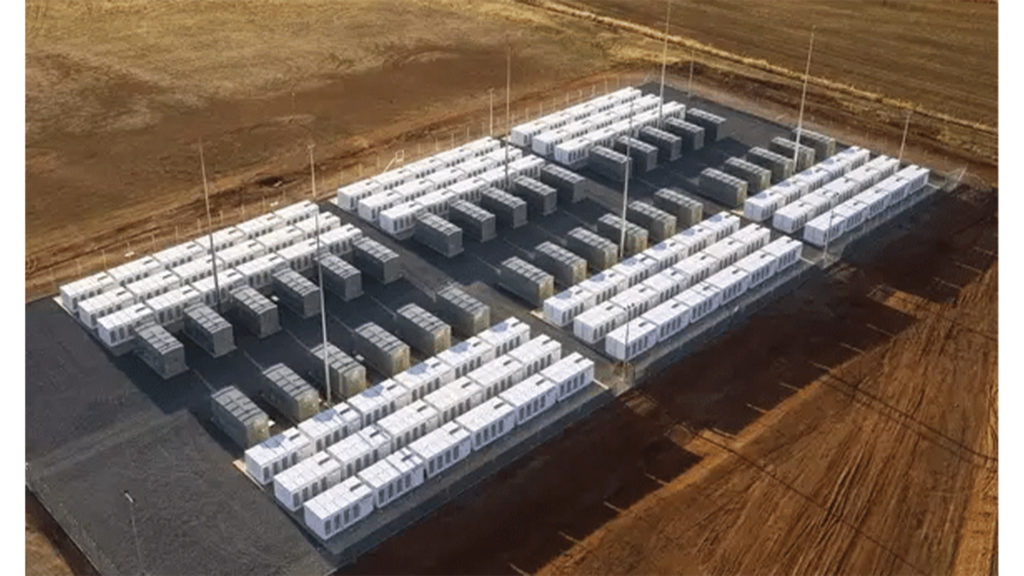
We need batteries, and batteries need nickel [and cobalt sulphate and graphene]. Almost 60 percent of the utility-scale batteries deployed in the U.S. last year were made by Samsung SDI and LG Chem, according to BNEF’s Sekine. Tesla’s gigantic battery factory can’t do it all. Tesla Inc. draws most of the public attention in the U.S., and its “gigafactory” in Nevada turns out batteries for home storage and utilities in addition to electric cars. The company’s battery-cell manufacturing partnership with Panasonic is currently in first place, with 105 gigawatt-hours of battery capacity either built or planned.
MATH:
We are building 100 new plants (80 Solar Plants, 20 Wind Plants).
100 Plants X $66 Million per battery equals a $6.6 Billion Bank Loan
NOTES:
- Oak Ridge National Laboratories has an aluminum-ion battery, we should work to have this replace Lithium-ion technology [or simply pay Elon Musk and use his – Tesla’s 100MW/129MWh Powerpack project in South Australia provides the same grid services as regular power plants, but cheaper, quicker, and with zero-emissions, through its battery system]
- People can already take Methane and Iron ore and create synthetic graphite, which might be helpful in our Battery Making Business (See few-layer graphene (FLG) and Hydrogen Fuel Manufacture).
Hydrogen
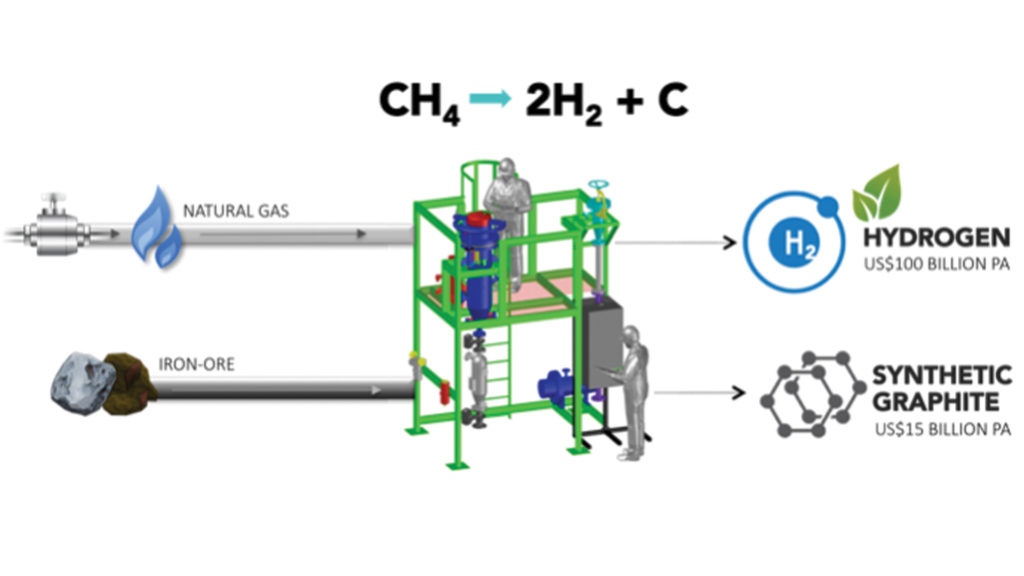
It takes 4.4 kWh’s to split one liter of water, producing 55.5 moles of hydrogen X 22.4 liters per mole = 1243.2 liters of hydrogen. Then it needs to be liquified at -400 degrees (too much volume as a gas), and it takes a lot of energy to liquify it, so while a fuel cell is theoretically can by 100% efficient, it is no better than 40% efficient yet, and very expensive to create. scientists are plugging away at it, though and are making the process easier all the time.
Nevertheless, several experts estimate that increased R&D funding for this may reduce capital costs by 0–24%, while production scale-up (just getting busy with it) will have an impact of 17–30%.
Some experts are backing Polymer Electrolyte Membrane Electrolysis (PEM).
The latest PEM technology will be used in Linz, in the form of 12 PEM modules with a total capacity of megawatts. In the future they will be able to produce 1,200 m3 of hydrogen an hour, a level currently unmatched. The hydrogen produced is also “green” (CO2-free, because, thanks to VERBUND, the electricity used for electrolysis is generated from renewable sources (hydropower and wind). They are also testing hydrogen’s suitability for use in the various process stages of steel production.
GO BIG
We would prefer our scientists to think even bigger and take all the methane from every source (human and animal and food waste, capture of flue gas emissions, etc) and turn it into methane, then turn that into Hydrogen and Carbon. With a little ingenuity (and maybe some Quicklime [CaO]), those tricky scientists might be able to get us some calcium carbonate (CaCO3), to use in our Cement business.
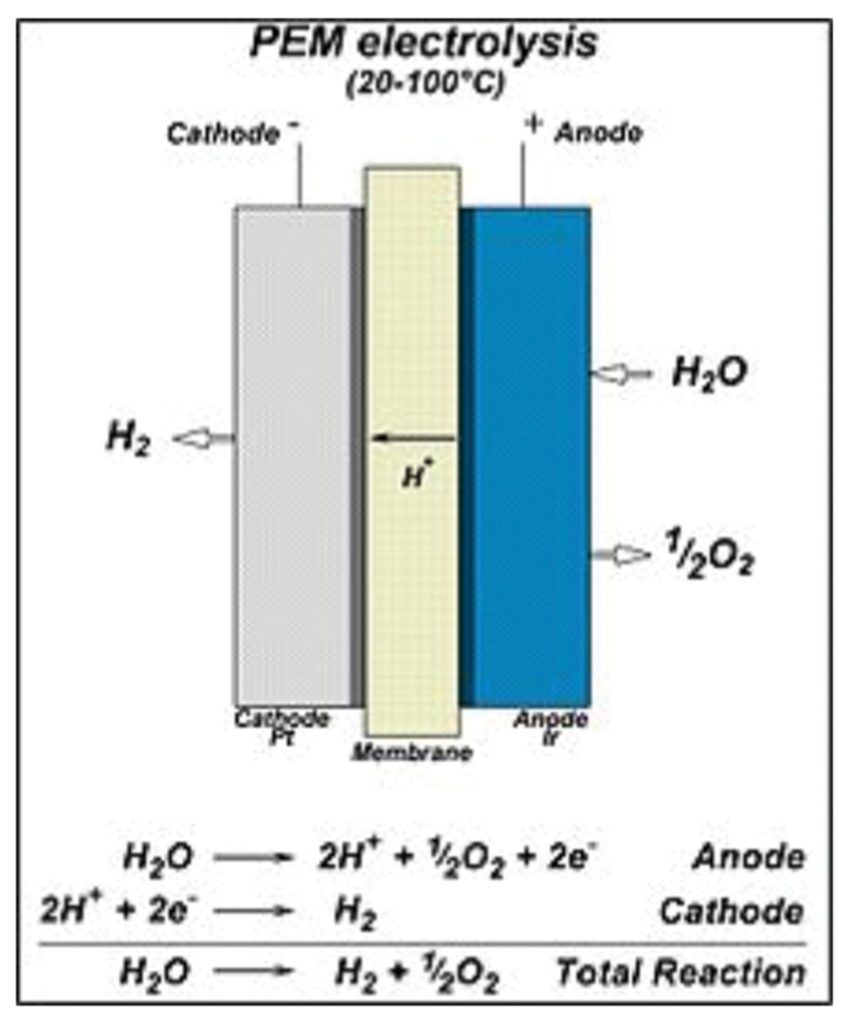
HYDROGEN FUELING STATIONS
We may need these more than Battery Charging Stations, as many Electric Vehicles are charged at home. If we go “all in”, We might build 30,000 of these across the US.
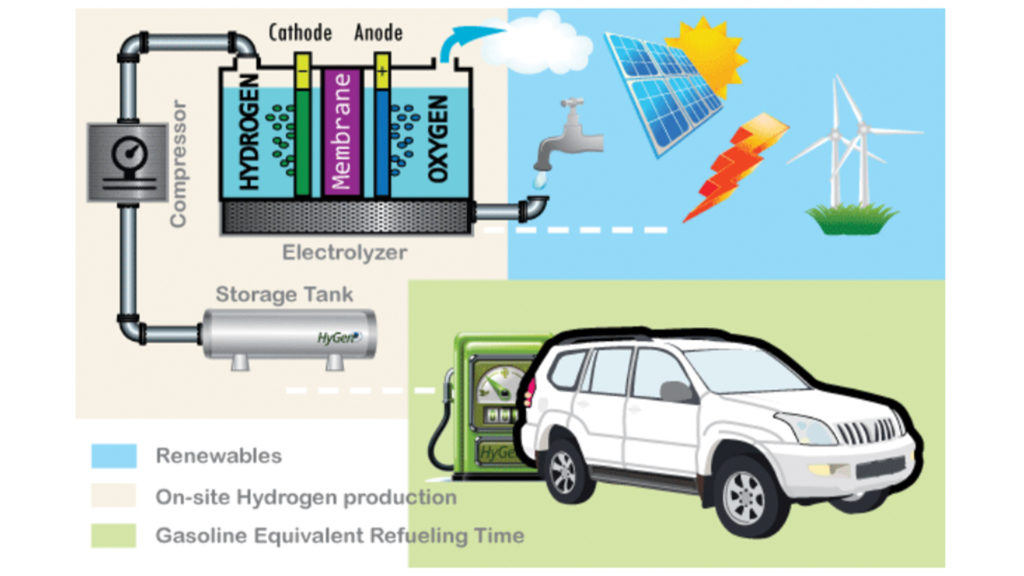
MATH:
A Station costs $2 Million X 30,000 of them = a $60 Billion Bank Loan
NOTE:
This fuel is recommended for larger vehicles like boats and railroad, both could shed the water by- product easily – look to using fuel for transport of Federal goods (timber, steel, construction materials, shipping to other countries of goods like tiny housing, omni-processors, etc).
HYDROGEN [OR METHANE] FROM WASTE
See Waste Management for Details, but Omni-processors cost $1.5 Million. Ours may be extra big and do more, so We will say they cost $2 Million. We need them in every Community, so $2 Million per Omni-processor X 3,271 Communities of 100,000 people = A $6.54 Billion Bank Loan.
Power Grid
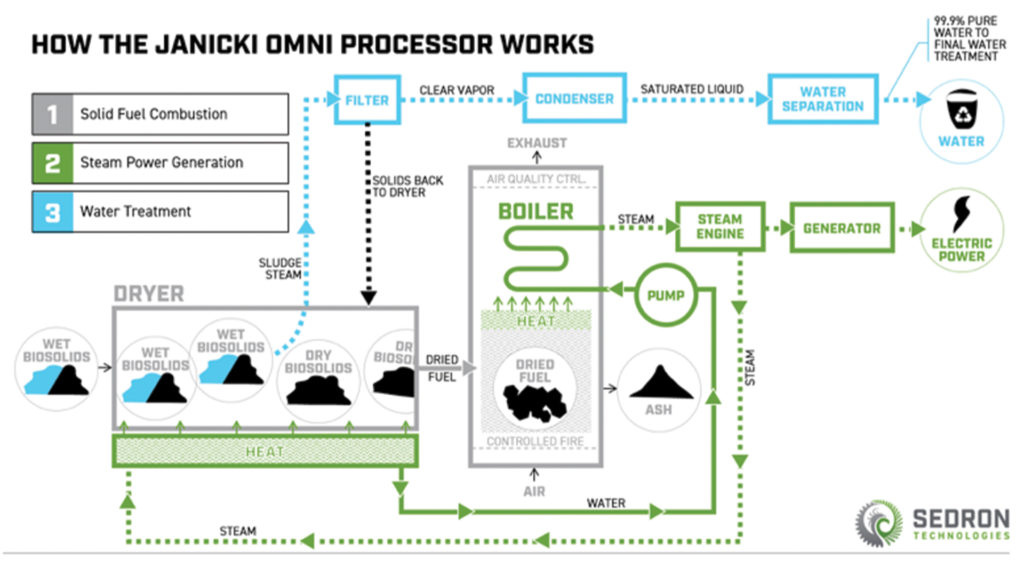
The U.S. electrical grid is the largest interconnected machine on Earth: 200,000 miles of high-voltage transmission lines and 5.5 million miles of local distribution lines, linking thousands of generating plants to factories, homes and businesses.
NOTES:
- Currently the grid has 500 separate owners. “There are participants who have a vested interest in the high price of electricity, not the low price of electricity.” – Douglas Gotham, an industry analyst at Purdue University.
- The Eastern Interconnection serves much of the United States east of the Great Plains. The Western Interconnection covers residents from the Great Plains to the Rockies and up and down the West Coast. And most of Texas has a grid of its own. Policymakers there shun ties with the other two grids in order to keep federal electric power regulation out of the Lone Star State.
- Equipment at the connection points is 30 to 40 years old and nearing the end of its useful life.
- High-voltage direct current (HVDC) technology is readily available and increasingly affordable, and could replace the old equipment to make long-distance electric power transfers between the eastern and western United States possible.
- Bloom and colleagues from Iowa State, Pacific Northwest National Labs, the Southwest Power Pool, the Midcontinent Independent System Operator, and the Western Area Power Administration built complex simulations in which solar power from the desert Southwest could help to meet peak electricity demand in the Northeast and then supports similar demand peaks a few hours later in the West.
- The study finds that “substantial value” exists in increasing the transfer capability between the grids. Maintaining the status quo of puny connections is the least desirable outcome. Second, cross-seam transmission has a substantial impact on the location, size, and type of wind and solar that could be developed in each region. Third, cross-seam transmission enables substantial energy and operating reserve sharing on a daily as well as on a seasonal basis. Fourth, there may be additional benefits as well as costs, including enhanced frequency response and resilience to extreme events. “Building national-scale transmission makes sense,” Bloom says.
- Connecting Grid will help find use for solar and wind power that gets wasted during low demand periods (battery storage will help solve this as well)
- Transmission siting in the United States is notoriously difficult, as proposed routes often require agreements with hundreds of landowners as well as approval by local, state, and federal agencies. Bloom says that designs that use HVDC lines could cut through some of the red tape as the transmission lines could be buried along existing railroad rights of way.
- For lines more than 300 to 500 miles long, DC outcompetes AC. After a certain distance, AC systems become more costly to build than DC and have larger power losses along the line because of issues such as higher resistance. “Using DC lines is a much better solution for moving power from big, remote wind or solar farms,” says Gregory Reed, director of the University of Pittsburgh’s Center for Energy and the Energy GRID Institute.
- Read More: It’s Time to Tie the U.S. Electric Grid Together, Says NREL Study Fortifying connections between three disparate grids could make renewable energy more widely available
The Quadrennial Energy Review [QER] focuses on modernizing the grid, expanding energy storage, and promoting interoperability with grid technology. The report looks at transmission, storage, and distribution infrastructure that the administration defines as: “The networks of pipelines, wires, storage, waterways, railroads, and other facilities that form the backbone of our energy system.” The report also addresses concerns over how to integrate renewable energy onto the grid.
When fully realized, the smart grid will perform as an automated energy-delivery network that monitors all power plants, customers, and individual appliances, and deliver real-time information and enable the near-instantaneous balance of supply and demand down to the device level. [See according to the U.S. Department of Energy]
All this calls upon the Internet of Things (IoT) model, which uses sensors and software to monitor a system in real time and continually report back about its findings. While the DOE says the smart grid is still several years away from realization, many utilities are installing smart meters for their customers and otherwise taking steps to bring real-rime systems monitoring to their energy networks (Read More: An Energy Grid With A Mind Of Its Own)
MATH
HVDC Transmission line costs $2.575 Million per mile. Our Government estimates it would cost $115.2 Billion to run HVDC lines from renewal sources like solar and wind, plus another 18.5 Billion in other repairs. We will start with these numbers and secure a $133.7 Billion Loan.
What We Have Learned
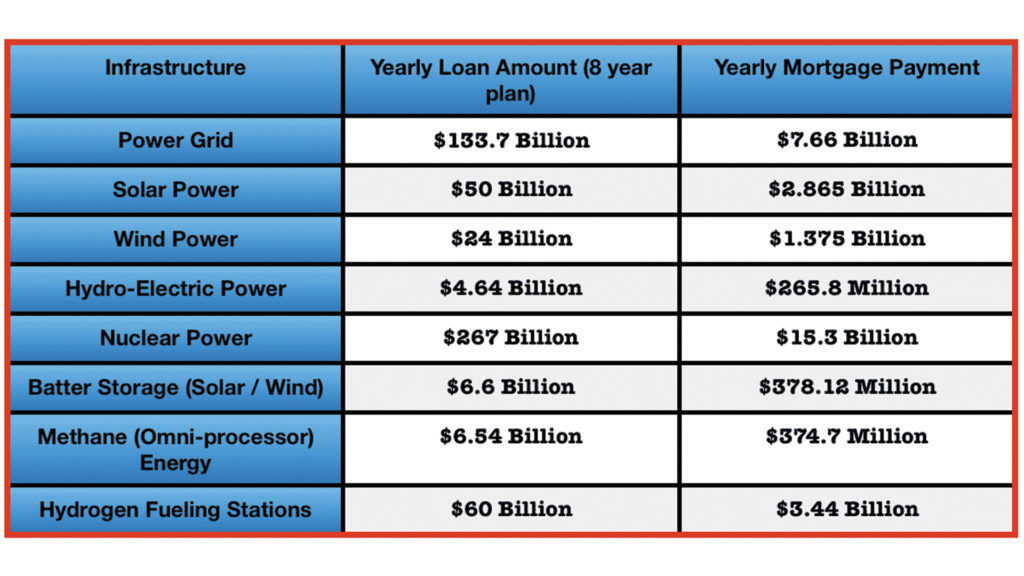
The US Energy Information Administration (EIA) says that in 2018, the average American spent 12.95 cents per KwH for electricity in the residential sector, though the overall rate throughout all sectors (transportation, Industry, Commercial) was 10.43 cents per KwH. Let’s say We charge everyone 10 cents per Kilowatt hour.
Solar Plants:
- 50 GW X 1 million (to convert to KwH) X 24 hours X 365 days X 22% efficiency X 10 cents = $9.636 Billion
- Cost to maintain panels is 1% of capital cost ($50 Billion) = $500 Million / yr
- $9.636 Billion – $500 Million = $9.136 Billion – $2.87 Billion (yearly Loan Payment) = $6.266 Billion Profit
Wind Plants:
- 16 GW X 1 million (to convert to KwH) X 24 hours X 365 days X 24% efficiency X 10 cents = $3.364 Billion
- Cost to Maintain is $48k per MW X 16,000 MW = $768 Million / yr
- $3.364 Billion – $768 Million = $2.596 Billion – $1.38 Billion (yearly Loan Payment) = $1.216 Billion Profit
Hydro Plants:
- 8 GW X 1 million (to convert to KwH) X 24 hours X 365 days X 90% efficiency X 10 cents = $6.31 Billion
- Cost to Maintain is $62.6k per 500 KW X 16,000 = $1 Billion / yr
- $6.31 Billion – $1 Billion = $5.3 Billion – $266 Million (yearly Loan Payment) =
$5 Billion Profit
TOTAL PROFIT / year = $12.5 Billion [totals from Solar / Wind / Hydro]- $7.66 Billion [to pay for the Power Grid each year] = $4.84 Billion in the first year (technically year 2)
Because we will build this amount every year…
- Year 3 = $9.68 Billion
- Year 4 = $14.52 Billion
- Year 5 = $19.36Billion
- Year 6 = $24.2 Billion
- Year 7 = $29.04 Billion
- Year 8 = $33.88 Billion
- Year 9 = $38.72 Billion
OVERALL PROFIT for 8 years = $174.24 Billion (average $21.78 Billion / yr)
TAKEAWAY:
The average American household uses nearly 11,000 KwH’s of electricity in a year, at an average of 12.95 cents per KwH. With our proposed uniform 10 cent charge per KwH, every household will spend $324 less a year on their Energy Bill each year.
We could charge even less, but We want people to use less of it, so there would be an equal argument to even raise the price. Even at this reasonable 10 cent rate, We could create a surplus to keep around for a “rainy day”, whether to help pay down the National Debt, or fund a government project (like Transportation, Education, Security, etc.)
(Read more about the Aluminum-ion Battery)
NOTE:
A baseline 1 gigawatt power plant with an uptime of 88% (typical for coal plants) will provide 1 GW x 365 x 24 x 0.88 = 7,700 gWh of energy over the year.
US uses perhaps 10,000 Gwh’s a day or 3.65 million Gigawatt hours in a year – this estimate seems to indicate that one giant 474 gigawatt power plant would cover us (think we better split that up, though)
Nuclear Notes
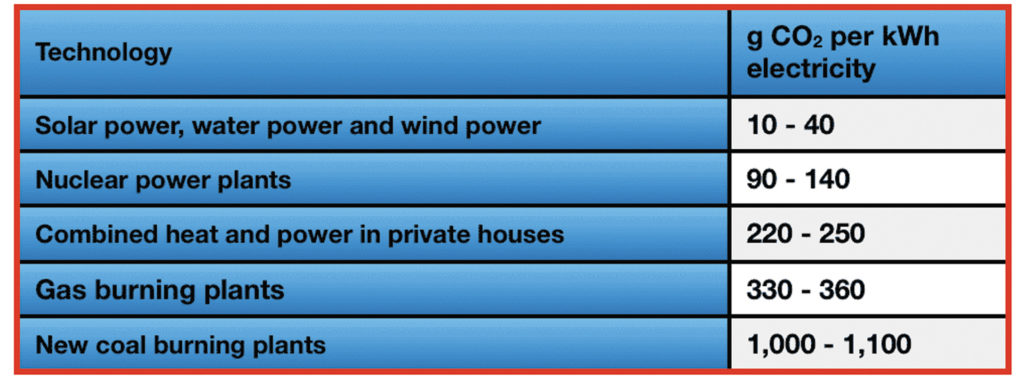
To calculate the amount of CO2 being released for a nuclear power plant, need to include construction, operation, maintenance and refurbishments, plus decommissioning and dismantling of the reactor. Of the same importance is the nuclear fuel: Recovery of uranium from the earth’s crust, extraction of uranium from ores, enrichment and chemical treatment, transportation as well as disposing of used fuel.
The world-wide reserves for Uranium are a very limited resource. It is estimated to last for about 50 to 70 years with the current demand. If additional nuclear reactors are built, the supply will grow even shorter.
A life cycle analyses (LCA) carried out by Jan Willem Storm van Leeuwen and Philip Smith came to the following result:
Electricity from atomic energy emits 90 to 140 g CO2 per kWh of electricity produced.
The relatively high range of uncertainty is due to the different grade of ores used. It depends on how rich the ores are that are used to obtain the Uranium. For poor ores, the higher value applies and for rich ores, the lower value applies.”
- https://www.stormsmith.nl/insight-items.html
- https://www.vox.com/energy-and-environment/2018/7/11/17555644/nuclear-power-energy-climate-decarbonization-renewables
- https://spectrum.ieee.org/energy/nuclear/terrapowers-nuclear-reactor-could-power-the-21st-century
As of today, there are more than 700,000 MT of depleted uranium in the United States [10]. This is a huge reserve that, with B&B reactor technology, can be directly used for energy production.
SMRs – some of the designs use a “breeder facility”, which helps to reduce the waste output. There are also numerous other SMRs in various states of completion too, from the 35 MWe KLT-40s which is under construction in Russia, to the Molten salt-based reactor still in the design stage in the UK.
SMRs also offer distinct safeguards, security and nonproliferation advantages.
Carbon Capture Technology
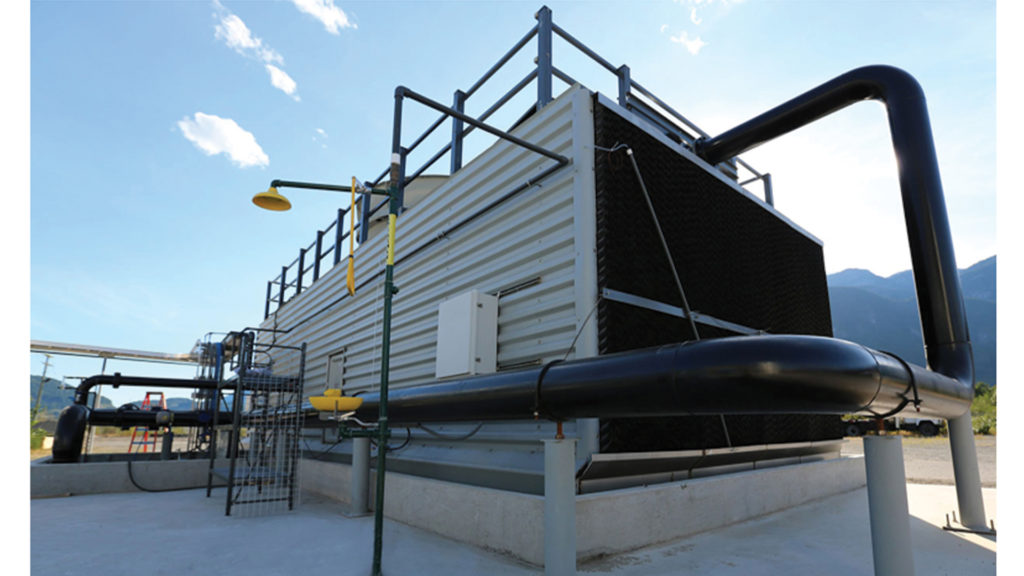
Harvard geoengineer David Keith has proven that direct air capture is doable on an industrial scale for $100 a ton.
Carbon Engineering, the company Keith founded in 2009 and that has Bill Gates’ support, has been capturing a ton of carbon dioxide every day since 2015 at its pilot plant north of Vancouver. Keith and his team have now come up with the first-ever detailed engineering design and cost analysis of a plant that would capture 1 million tons of carbon dioxide a year.
Carbon Engineering uses a bank of giant fans to draw ambient air and push it through an aqueous solution that reacts with carbon dioxide. Heat and known chemical reactions separate the CO2 molecules. The company combines it with hydrogen produced from water electrolysis to create liquid fuels that can be used in today’s trucks and aircraft engines. They started making these fuels at the plant in December.
Keith acknowledges that making carbon dioxide an ingredient in fuel doesn’t help to reduce the overall amount of carbon dioxide in the atmosphere. But because the carbon-neutral fuel replaces fossil fuels that would add more planet-warming gas to the atmosphere, it would make a large dent in transportation-related CO2 emissions.
Hydrogen Production and Distribution
Once separated, hydrogen can be used along with oxygen from the air in a fuel cell to create electricity through an electrochemical process.
Production
Hydrogen can be produced from diverse, domestic resources including fossil fuels, biomass, and water electrolysis with electricity. The environmental impact and energy efficiency of hydrogen depends on how it is produced. Some projects are underway to decrease costs associated with hydrogen production (PDF)
There are a number of ways to produce hydrogen:
- Natural Gas Reforming/Gasification: Synthesis gas, a mixture of hydrogen, carbon monoxide, and a small amount of carbon dioxide, is created by reacting natural gas with high-temperature steam. The carbon monoxide is reacted with water to produce additional hydrogen. This method is the cheapest, most efficient, and most common. Natural gas reforming using steam accounts for the majority of hydrogen produced in the United States annually. A synthesis gas can also be created by reacting coal or biomass with high-temperature steam and oxygen in a pressurized gasifier, which is converted into gaseous components—a process called gasification. The resulting synthesis gas contains hydrogen and carbon monoxide, which is reacted with steam to separate the hydrogen.
- Electrolysis: An electric current splits water into hydrogen and oxygen. If the electricity is produced by renewable sources, such as solar or wind, the resulting hydrogen will be considered renewable as well, and has numerous emissions benefits. Power-to-hydrogen projects are taking off, where excess renewable electricity, when it’s available, is used to make hydrogen through electrolysis.
- Renewable Liquid Reforming: Renewable liquid fuels, such as ethanol, are reacted with high-temperature steam to produce hydrogen near the point of end use.
- Fermentation: Biomass is converted into sugar-rich feedstocks that can be fermented to produce hydrogen.
A number of hydrogen production methods are in development:
- High-Temperature Water Splitting: High temperatures generated by solar concentrators or nuclear reactors drive chemical reactions that split water to produce hydrogen.
- Photobiological Water Splitting: Microbes, such as green algae, consume water in the presence of sunlight, producing hydrogen as a byproduct.
- Photoelectrochemical Water Splitting: Photoelectrochemical systems produce hydrogen from water using special semiconductors and energy from sunlight.
The major hydrogen-producing states are California, Louisiana, and Texas. Today, almost all of the hydrogen produced in the United States is used for refining petroleum, treating metals, producing fertilizer, and processing foods.
The primary challenge for hydrogen production is reducing the cost of production technologies to make the resulting hydrogen cost competitive with conventional transportation fuels. Government and industry research and development projects are reducing the cost as well as the environmental impacts of hydrogen production technologies. Learn more about hydrogen production from the Fuel Cell Technologies Office and the National Renewable Energy Laboratory.
Distribution
Most hydrogen used in the United States is produced at or close to where it is used—typically at large industrial sites. The infrastructure needed for distributing hydrogen to the nationwide network of fueling stations required for the widespread use of fuel cell electric vehicles still needs to be developed. The initial rollout for vehicles and stations focuses on building out these distribution networks, primarily in southern and northern California.
Currently, hydrogen is distributed through three methods:
- Pipeline: This least-expensive way to deliver large volumes of hydrogen is limited—because there is only about 700 miles of U.S. pipelines for hydrogen delivery currently available. These pipelines are located near large petroleum refineries and chemical plants in Illinois, California, and the Gulf Coast.
- High-Pressure Tube Trailers: Transporting compressed hydrogen gas by truck, railcar, ship, or barge in high-pressure tube trailers is expensive and used primarily for distances of 200 miles or less.
- Liquefied Hydrogen Tankers: Cryogenic liquefaction is a process that cools the hydrogen to a temperature where it becomes a liquid. Although the liquefaction process is expensive, it enables hydrogen to be transported more efficiently (when compared with using high-pressure tube trailers) over longer distances by truck, railcar, ship, or barge. If the liquefied hydrogen is not used at a sufficiently high rate at the point of consumption, it boils off (or evaporates) from its containment vessels. This fact requires that the hydrogen delivery and consumption rates are carefully matched.
- Creating an infrastructure (for hydrogen distribution and delivery (PDF)
- To thousands of future individual fueling stations presents many challenges. Because hydrogen contains less energy per unit volume than all other fuels, transporting, storing, and delivering it to the point of end-use is more expensive on a per gasoline gallon equivalent (per-GGE) basis. Building a new hydrogen pipeline network involves high initial capital costs, and hydrogen’s properties present unique challenges to pipeline materials and compressor design. However, because hydrogen can be produced from a wide variety of resources, regional or even local hydrogen production can maximize use of local resources and minimize distribution challenges.
- There are tradeoffs between centralized and distributed production to consider. Producing hydrogen centrally in large plants cuts production costs but boosts distribution costs. Producing hydrogen at the point of end-use—at fueling stations, for example—cuts distribution costs but increases production costs because of the cost to construct on-site production capabilities.
- Government and industry research and development projects are overcoming the barriers to efficient hydrogen distribution. Learn more about hydrogen distribution from the Fuel Cell Technologies Office.
Fuel Cell Vehicles
Fuel cell vehicles (FCVs) have the potential to significantly reduce our dependence on foreign oil and lower harmful emissions that contribute to climate change. FCVs run on hydrogen gas rather than gasoline and emit no harmful tailpipe emissions. Several challenges must be overcome for them to be competitive with conventional vehicles, but their potential benefits are substantial.
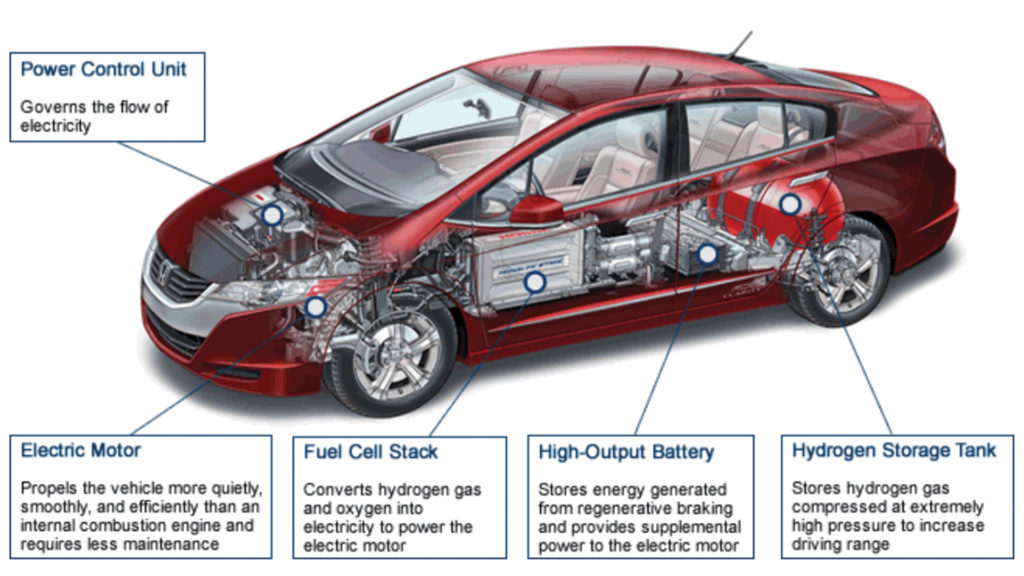
Fuel cell vehicles (FCVs) have the potential to significantly reduce our dependence on foreign oil and lower harmful emissions that contribute to climate change. FCVs run on hydrogen gas rather than gasoline and emit no harmful tailpipe emissions. Several challenges must be overcome for them to be competitive with conventional vehicles, but their potential benefits are substantial.
A Look Inside
FCVs look like conventional vehicles but use cutting edge technologies. The heart of the FCV is the fuel cell stack. The stack converts hydrogen gas stored onboard with oxygen from the air into electricity, which powers the vehicle’s electric motor. The major components of a typical FCV are illustrated below.
Hydro Power
The most common type of hydroelectric power plant uses a dam on a river to store water in a reservoir. Water released from the reservoir flows through a turbine, spinning it, which in turn activates a generator to produce electricity. But hydroelectric power doesn’t necessarily require a large dam. Some hydroelectric power plants just use a small canal to channel the river water through a turbine.
Another type of hydroelectric power plant – called a pumped storage plant – can even store power. The power is sent from a power grid into the electric generators. The generators then spin the turbines backward, which causes the turbines to pump water from a river or lower reservoir to an upper reservoir, where the power is stored. To use the power, the water is released from the upper reservoir back down into the river or lower reservoir. This spins the turbines forward, activating the generators to produce electricity.
- A small or micro-hydroelectric power system can produce enough electricity for a home, farm, or ranch.
- The ocean can produce two types of energy: thermal energy from the sun’s heat, and mechanical energy from the tides and waves.
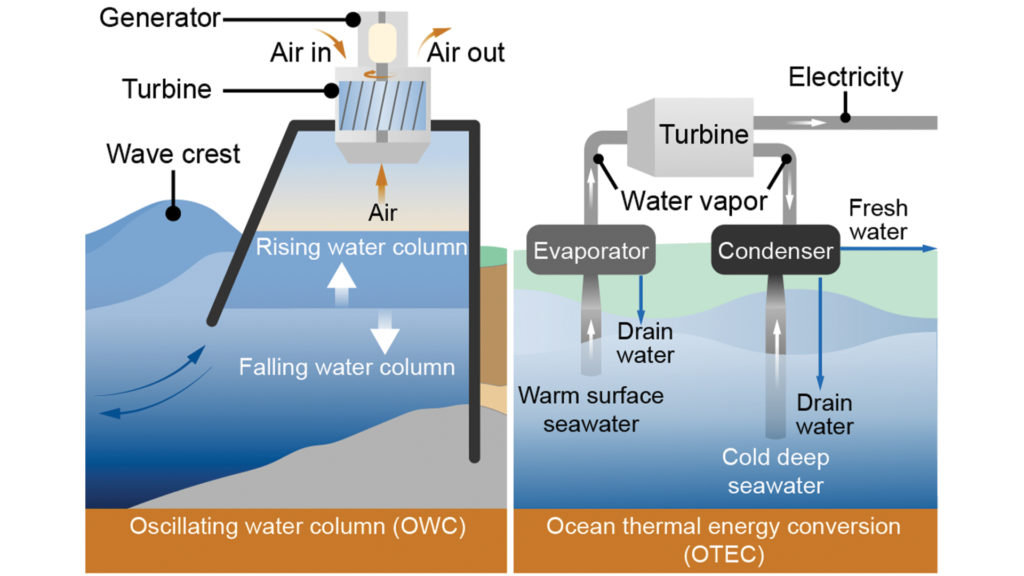
Oceans cover more than 70% of Earth’s surface, making them the world’s largest solar collectors. The sun’s heat warms the surface water a lot more than the deep ocean water, and this temperature difference creates thermal energy. Just a small portion of the heat trapped in the ocean could power the world.
- Ocean thermal energy is used for many applications, including electricity generation. There are three types of electricity conversion systems: closed-cycle, open-cycle, and hybrid. Closed-cycle systems use the ocean’s warm surface water to vaporize a working fluid, which has a low-boiling point, such as ammonia. The vapor expands and turns a turbine. The turbine then activates a generator to produce electricity. Open-cycle systems actually boil the seawater by operating at low pressures. This produces steam that passes through a turbine/generator. And hybrid systems combine both closed-cycle and open-cycle systems.
- Ocean mechanical energy is quite different from ocean thermal energy. Even though the sun affects all ocean activity, tides are driven primarily by the gravitational pull of the moon, and waves are driven primarily by the winds. As a result, tides and waves are intermittent sources of energy, while ocean thermal energy is fairly constant. Also, unlike thermal energy, the electricity conversion of both tidal and wave energy usually involves mechanical devices.
- A barrage (dam) is typically used to convert tidal energy into electricity by forcing the water through turbines, activating a generator. For wave energy conversion, there are three basic systems: channel systems that funnel the waves into reservoirs; float systems that drive hydraulic pumps; and oscillating water column systems that use the waves to compress air within a container. The mechanical power created from these systems either directly activates a generator or transfers to a working fluid, water, or air, which then drives a turbine/generator.